Polycrystalline silicon, also known as polysilicon or multi-crystalline silicon, is a vital raw material used in the solar photovoltaic and electronics industries. As the demand for renewable energy and advanced electronic devices continues to grow, understanding the polysilicon manufacturing process is crucial for appreciating the properties, cost, and environmental impact of the end products. This comprehensive article provides an in-depth look at the complex journey from raw quartz sand to high-purity polysilicon, focusing on the key steps, technologies, and challenges involved.
What is Polycrystalline Silicon?
Polycrystalline silicon is a high-purity form of silicon consisting of multiple small silicon crystals. It is the primary raw material used in the production of solar cells and various electronic devices, such as integrated circuits and MEMS (Micro-Electro-Mechanical Systems). The manufacturing process of polysilicon involves several complex steps, starting with the extraction and purification of raw materials and ending with the production of high-purity polysilicon chunks or granules.
Raw Material Extraction and Purification
The journey of polysilicon begins with its primary raw material: quartz sand. Quartz, composed of silicon dioxide (SiO2), is one of the most abundant minerals in the Earth’s crust. However, the silicon found in nature is not pure enough for direct use in solar cells or electronic devices. It must undergo a series of purification processes to reach the required purity levels.
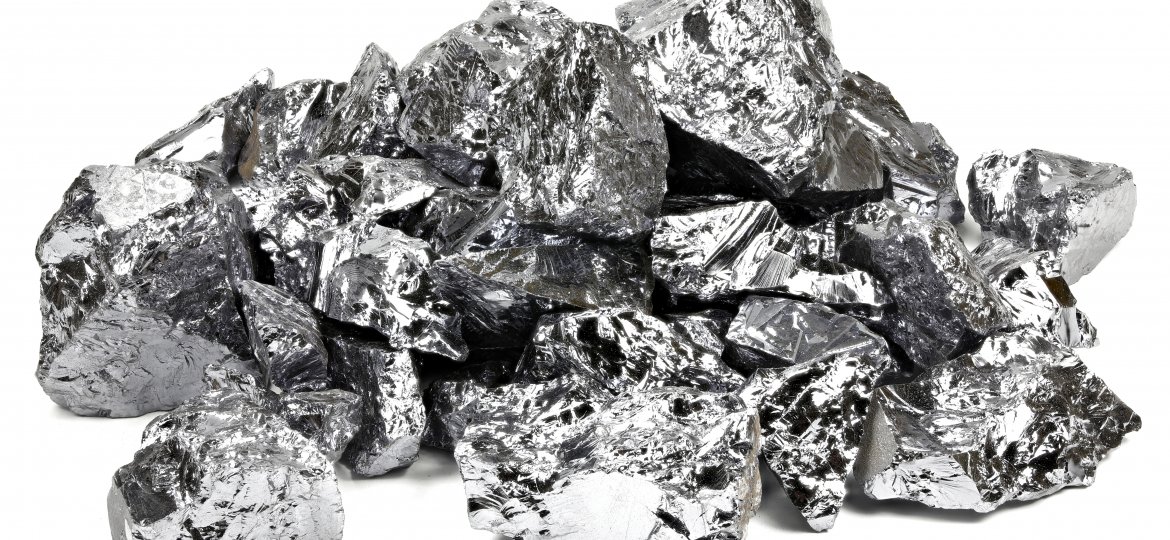
The first step involves heating raw quartz sand in an electric arc furnace along with carbon sources like coal, coke, or wood chips. At temperatures around 2000°C, the carbon reduces the silicon dioxide to produce metallurgical-grade silicon (MGS) with a purity of approximately 98-99%.
To further purify the MGS, it is ground into a fine powder and fed into a fluidized bed reactor (FBR) along with hydrogen and hydrochloric acid (HCl) gas. Inside the FBR, a series of chemical reactions take place, converting the MGS into trichlorosilane (SiHCl3) gas. This step removes impurities like boron, phosphorus, and metal ions that could negatively impact the performance of the final polysilicon product.
The trichlorosilane gas then undergoes fractional distillation to separate it from byproducts and impurities. The purified trichlorosilane serves as the starting point for polysilicon production via the widely used Siemens process or the more recently developed fluidized bed reactor technology.
Polysilicon Production: The Siemens Process
The Siemens process, named after its inventor, has been the dominant method for producing high-purity polysilicon for several decades. In this process, purified trichlorosilane gas and hydrogen are introduced into a Siemens reactor, which contains high-purity silicon filaments or rods heated to temperatures between 1000-1200°C.
As the gas mixture flows over the heated filaments, it undergoes a chemical vapor deposition (CVD) reaction. The trichlorosilane molecules decompose, depositing pure silicon atoms onto the filaments while releasing hydrogen chloride gas. Over time, the silicon builds up on the filaments, causing them to grow into large, cylindrical polysilicon rods.
The Siemens process is highly energy-intensive, requiring significant amounts of electricity to maintain the high temperatures needed for the CVD reaction. To minimize contamination, the reactor chambers are typically made of high-purity quartz glass and operate under tightly controlled conditions.
Once the polysilicon rods have reached the desired size, they are removed from the reactor, cooled, and broken into chunks of varying sizes. The resulting polysilicon has a purity of 9N to 11N (99.9999999% to 99.999999999% pure), depending on the intended application.
Emerging Technology: Fluidized Bed Reactors
In recent years, fluidized bed reactor (FBR) technology has emerged as a promising alternative to the traditional Siemens process for polysilicon production. FBR technology offers several advantages, including lower energy consumption, continuous operation, and the ability to produce granular polysilicon directly.
In the FBR process, a bed of silicon seed particles is suspended in an upward flow of hydrogen and silicon-containing gas, typically silane (SiH4) or trichlorosilane. As the gas mixture passes through the bed, the silicon-containing molecules decompose and deposit onto the seed particles, causing them to grow. The resulting granular polysilicon is continuously removed from the bottom of the reactor while new seed particles are added at the top.
One of the key challenges in FBR technology is maintaining a uniform temperature and gas distribution throughout the reactor to ensure consistent polysilicon quality. Companies like REC Silicon and GCL-Poly have invested heavily in developing and scaling up FBR technology, aiming to reduce production costs and improve the environmental footprint of polysilicon manufacturing.
Polysilicon Quality and Applications
The purity and crystal structure of polysilicon have a significant impact on its suitability for various applications. In the solar photovoltaic industry, which consumes a majority of the global polysilicon supply, two main types of polysilicon are used: solar-grade and electronic-grade.
Solar-grade polysilicon, typically with a purity of 6N to 9N, is used to produce multi-crystalline and mono-crystalline silicon wafers for solar cells. While solar-grade polysilicon has a lower purity compared to electronic-grade, it is more cost-effective and still provides sufficient performance for solar energy conversion.
Electronic-grade polysilicon, with a purity of 9N to 11N, is used in the semiconductor industry to manufacture integrated circuits, MEMS devices, and other high-performance electronic components. The higher purity levels are necessary to ensure reliable device performance and minimize defects.
Environmental Impact and Sustainability
The polysilicon manufacturing process, particularly the Siemens process, is energy-intensive and has a significant environmental footprint. The high temperatures required for the CVD reaction in the Siemens process are typically achieved using electricity generated from fossil fuels, leading to substantial greenhouse gas emissions.
Moreover, the production of polysilicon involves the use of hazardous chemicals like hydrochloric acid and trichlorosilane, which pose risks to human health and the environment if not properly managed. Stringent regulations and safety protocols are in place to minimize these risks, but concerns remain about the long-term sustainability of polysilicon production.
To address these challenges, the polysilicon industry is actively exploring ways to reduce its environmental impact. Some key initiatives include:
- Adopting renewable energy sources like solar and wind power to reduce reliance on fossil fuels.
- Improving energy efficiency through process optimizations and the development of new technologies like FBR.
- Implementing closed-loop systems to recycle and reuse chemicals, minimizing waste and reducing the need for virgin raw materials.
- Investing in research and development to find alternative, more sustainable production methods and materials.
As the demand for solar energy and electronic devices continues to grow, it is crucial for the polysilicon industry to prioritize sustainability and work towards minimizing its environmental footprint.
Future Outlook and Challenges
The global polysilicon market is expected to experience significant growth in the coming years, driven by the increasing adoption of solar energy and the rapid expansion of the electronics industry. However, the industry also faces several challenges that must be addressed to ensure its long-term sustainability and competitiveness.
One of the main challenges is the need to reduce production costs while maintaining high product quality. The development of new technologies like FBR and process optimizations will play a crucial role in achieving this goal. Additionally, the industry must work to diversify its supply chain and reduce its reliance on a few key producers to mitigate risks associated with supply disruptions and price volatility.
Another critical challenge is the need to address the environmental and social impacts of polysilicon production. This includes reducing greenhouse gas emissions, minimizing the use of hazardous chemicals, and ensuring safe and fair working conditions for employees. The industry must also work to increase transparency and engage with stakeholders to build trust and support for its operations.
Looking to the future, the polysilicon industry has the potential to play a vital role in the transition to a more sustainable and low-carbon economy. By continuously innovating, improving efficiency, and prioritizing sustainability, the industry can help enable the widespread adoption of renewable energy and advanced electronic technologies that will shape our world in the coming decades.
Frequently Asked Questions
Polycrystalline silicon consists of multiple small silicon crystals, while monocrystalline silicon is composed of a single continuous crystal. Monocrystalline silicon generally has higher purity and better electrical properties, but it is more expensive to produce compared to polycrystalline silicon.
Polycrystalline silicon is primarily used in the solar photovoltaic industry to produce solar cells and in the electronics industry to manufacture integrated circuits, MEMS devices, and other high-performance electronic components.
The purity of polycrystalline silicon directly impacts its performance in various applications. Higher purity levels result in better electrical properties, increased efficiency, and reduced defects. Solar-grade polysilicon (6N-9N purity) is suitable for solar cells, while electronic-grade polysilicon (9N-11N purity) is required for high-performance electronic devices.
Conclusion
The polycrystalline silicon manufacturing process is a complex and energy-intensive journey that transforms abundant raw materials like quartz sand into a high-purity, versatile material essential for the solar photovoltaic and electronics industries. From the initial purification of metallurgical-grade silicon to the advanced Siemens process and emerging fluidized bed reactor technology, each step plays a crucial role in determining the quality, cost, and environmental impact of the final polysilicon product.
As the demand for polysilicon continues to grow, driven by the increasing adoption of solar energy and the rapid expansion of the electronics industry, it is essential for manufacturers to prioritize sustainability, innovation, and operational excellence. By continuously improving production processes, investing in research and development, and working to minimize environmental impacts, the polysilicon industry can help enable a more sustainable and technologically advanced future for all.